Research
Many physiological processes such as cell division or cell migration include cellular shape changes. Like all solid-state matter, cells resist shape changes through their material stiffness. Understanding this material stiffness is therefore pivotal for our understanding of biological processes that rely on cell shape changes. Our group works on the development of theoretical and experimental tools for the quantification of active material properties of cells and tissues. Furthermore, we want to pinpoint how these active material properties emerge from underlying molecular constituents. Some exemplary former projects that illustrate this mission are described below.
Tension-sensing cross-linkers in the actin cytoskeleton
The actin cytoskeleton is a living material that constitutes a key structure for cellular mechanics and cellular force generation. Therefore, the actin cytoskeleton plays a major role in cell shape regulation, cell migration and tissue organization. In the past decades, actin cross-linkers have been shown to be sensitive regulators of viscoelastic mechanics of the actin cytoskeleton. The biological importance of actin cross-linkers in cytoskeletal function is also reflected in the fact that actin cross-linker mutations and expression changes have been associated with a wide spectrum of diseases such as cancer, skeletal dysplasia, renal diseases and many others. This underscores the high importance of actin cross-linkers in the physiological function of cells.
In our group, we investigate experimentally how the binding dynamics of actin cross-linkers is modified by mechanical tension in the actin cortex and how this tension-sensing of cross-linkers feedbacks on the emergent mechanical properties of the actin cortex.
Furthermore, we explore how tension-sensitive cross-linkers influence the proficiency of active material to form self-organized patterns.
Find out more about our experimental work on the mechanosensitivity of α-actinin-4 our recent publication (reference 2).
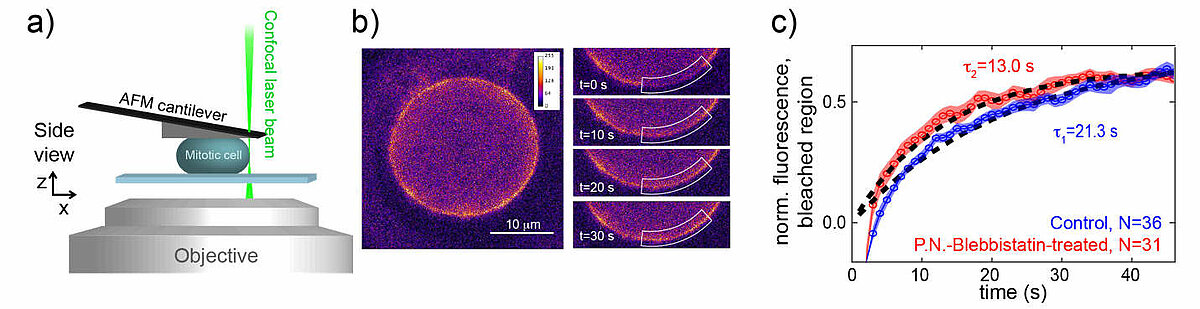
Cortex mechanics and its effect on mitotic rounding in cancer cells
At the onset of mitosis, many animal cells deform to acquire an approximately spherical shape. This process has been termed mitotic rounding. This mitotic rounding ensures space for the correct positioning of the mitotic spindle. It is a requirement for timely and successful cell division. Mitotic rounding relies on mechanical deformation of surrounding tissue and corresponding forces are emanating, at least partly, from the contractility of the cellular actin cortex.
Our group investigates actin cortex mechanics in relation to mitotic rounding, using a combination of a cell confinement assay and 3D tumor spheroid studies. In particular, we study how epithelial mesenchymal transition (EMT), a hallmark of cancer progression and metastasis, affects the mechanics and contractility of the actin cortex. Furthermore, we study the effects of EMT on mitotic rounding and cell proliferation. Details about our work on EMT in relation to cortex mechanics and mitotic rounding can be found in references 1, 3, 7.
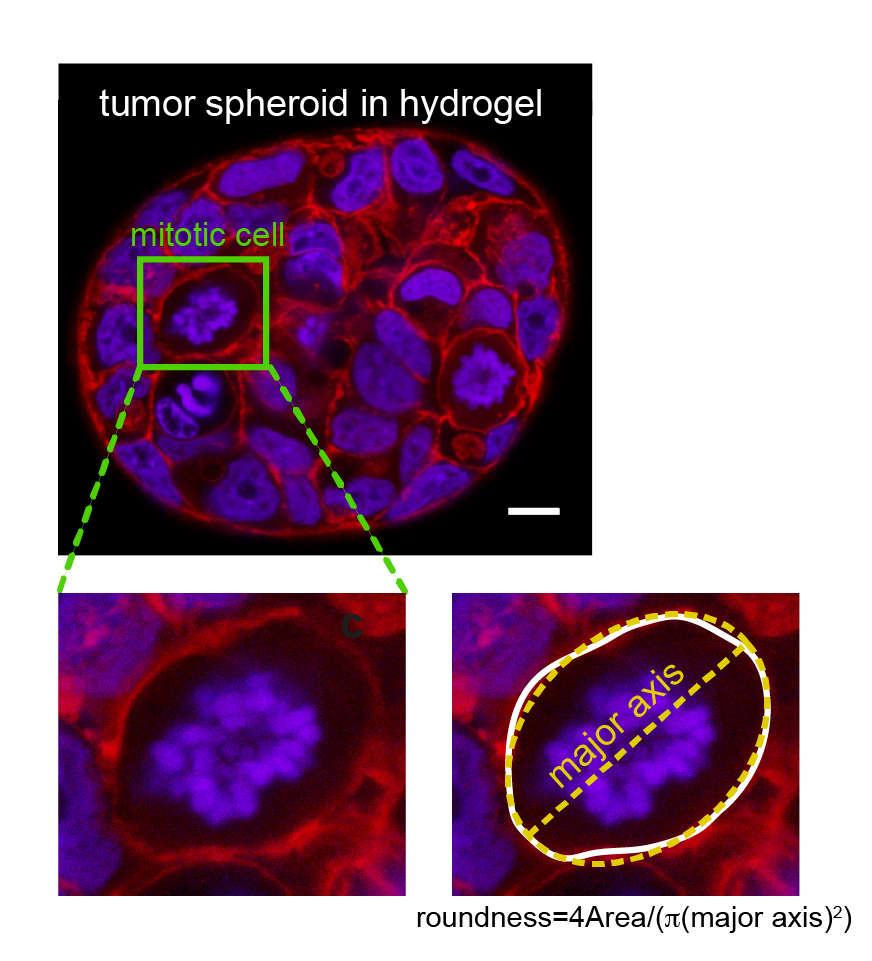
Rheological measurements on the actin cortex by a cell confinement assay
The cell cortex is a cytoskeletal meshwork of polymerized actin proteins that mechanically supports the inner side of the cellular plasma membrane. The cortex is constantly renewing itself through cycles of polymerization and depolymerization. Due to this turnover, it has long been hypothesized that the cortex behaves fluid-like on timescales larger than its turnover timescale. However, it was difficult to test this hypothesis experimentally as the cortex is connected to other cellular structures that generally contribute to the cellular force response in cell-mechanical probing. We have established a cell confinement assay using atomic force microscopy (AFM) that dominantly probes the cellular cortex mechanically. In this assay, we dynamically compress (non-adherent) cells between parallel plates thereby stretching the overall cell surface and thus the cortical layer of the cell. We could extract a frequency-dependent complex elastic modulus that characterizes the mechanical resistance of the cortex with respect to area dilation. Indeed, we find that the cell cortex starts to behave dominantly fluid-like for frequencies lower than ~0.01 Hz.
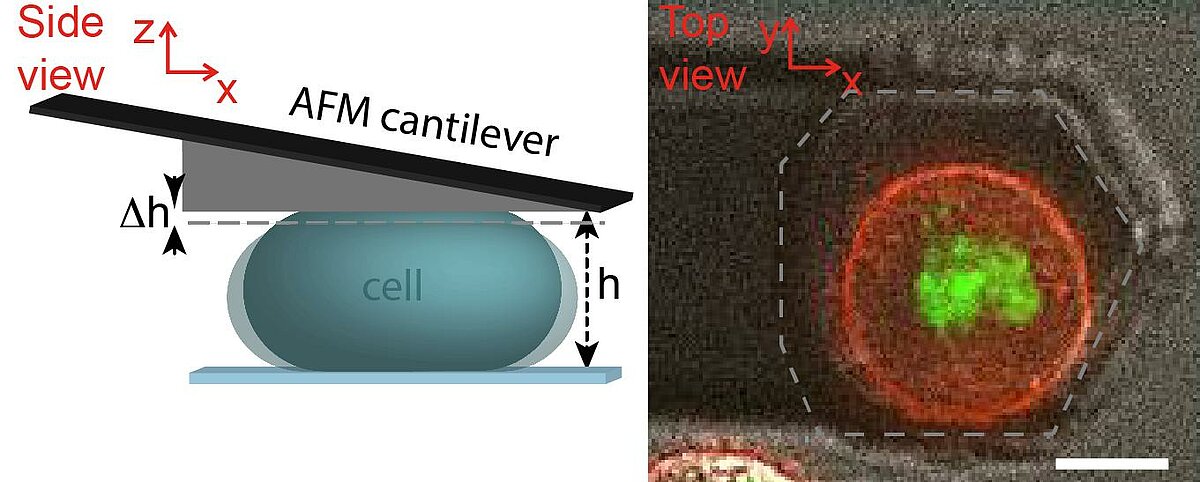
Cellular rheology exhibits a characteristic timescale, which marks the onset of fluidity of the cellular cortex. Still, cortical rheology is not captured by a single relaxation timescale as in the case of a simple Maxwell model. Also, a power law as commonly found in the rheology of adherent cells does not capture cortical rheology as it is devoid of a characteristic timescale. We have established a new rheological model that captures cortical rheology. This model is defined through a constant relaxation spectrum up to a cut-off timescale. This cut-off timescale corresponds to a slowest relaxation mode in the material. Our new rheological model reconciles key features of the commonly used Maxwell and power law models, because it exhibits a (slowest) characteristic timescale but also a continuum of smaller relaxation timescales. Interestingly, we find that that the slowest relaxation timescale of the cortex is similar to turnover times of cortical cross-linkers. This finding strengthens the idea that cortex fluidization stems from cortical turnover.
Details about our work on actin cortex mechanics can be found in our publications (references 1, 4, 6).
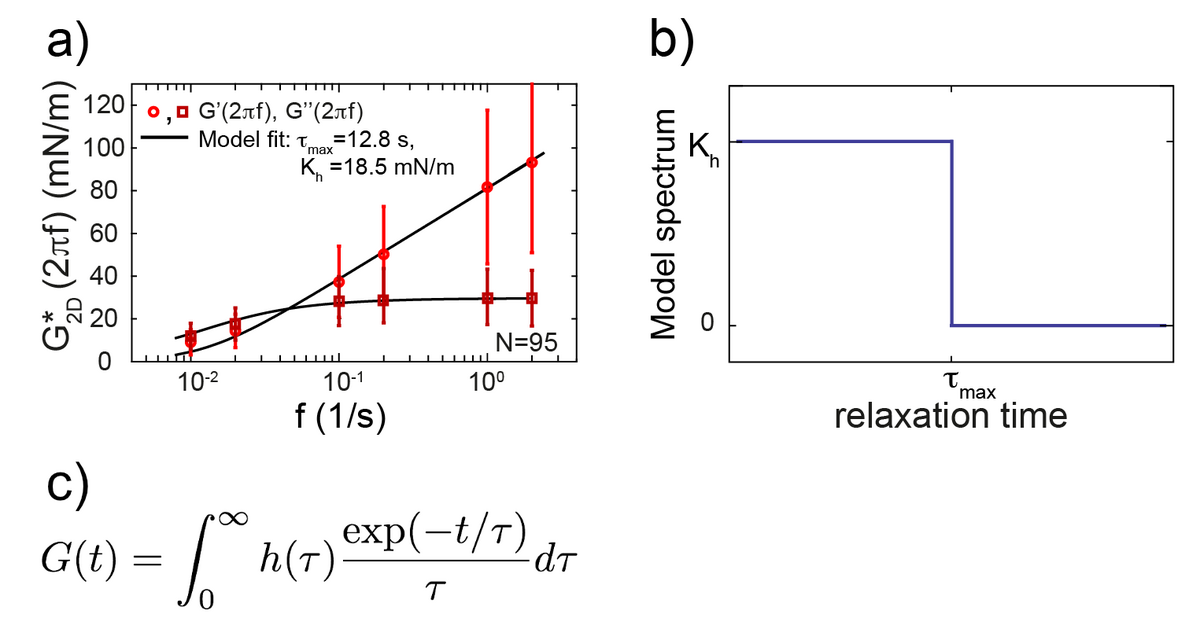